By Caleb O’Brien | MU Bond Life Sciences Center
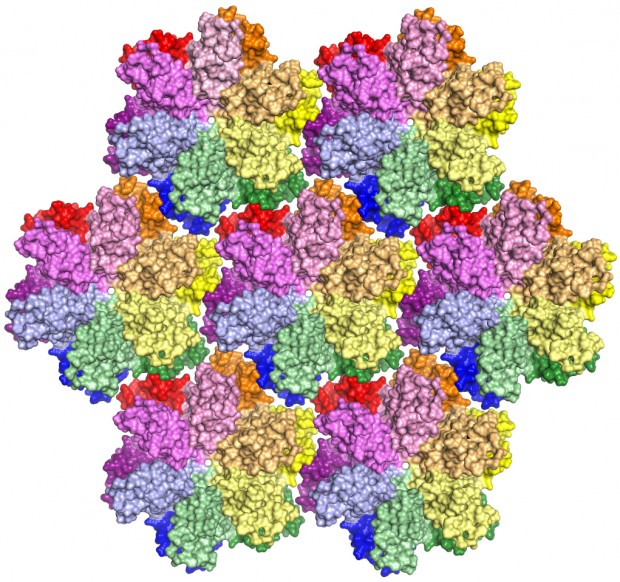
Seeing the whole picture can mean a lot when it comes to figuring out HIV.
Researchers at the University of Missouri Bond Life Sciences Center are gaining a clearer idea of what a key protein in HIV looks like, which will help explain the flexible protein’s vital role in the virus life cycle.
The protein the researchers imaged is a building block that forms the virus’ capsid, a protective shell surrounding the virus’ genes. The journal Science published their findings online June 4.
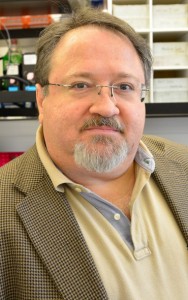
“The capsid acts as an invisibility cloak that hides the virus’ genetic information, the genome, while it is being copied in a hostile environment for the virus,” said Stefan Sarafianos, a virologist at Bond LSC and lead author of the study. “Fine-tuned capsid stability is critical for successful infection: too stable a capsid shell and the cargo is never delivered properly; not stable enough and the contents are detected by our immune defenses, triggering an antiviral response. Capsid stability is a key to the puzzle, and to solve it you have to understand its structure.”
This is the most complete model yet of an HIV-1 capsid protein. In a virus, the protein combines in groups of five or six — called pentamers and hexamers, respectively — that assemble into a mosaic that forms the capsid shell. Roughly 1,500 copies of the protein, grouped into about 250 hexamers and 12 pentamers, comprise the capsid.
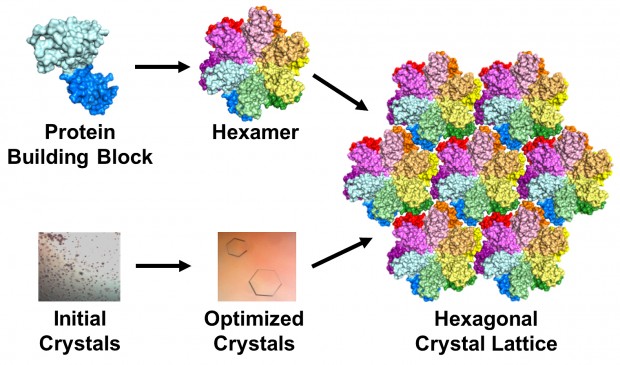
HIV, or human immunodeficiency virus, is the retrovirus that leads to AIDS — acquired immunodeficiency syndrome. Roughly 1.2 million people live with HIV in the United States, according to the Centers for Disease Control and Prevention. Globally, about 35 million people were living with HIV in 2013.
A lucky break
Over the years, scientists have employed various techniques and tricks to figure out the structure of the capsid protein. But until now, the clearest image had been made of a mutated version of the protein. It was a compromise: the mutation made the protein stable enough that the scientists could get a good snapshot, but they couldn’t see the detailed interactions between hexamers.
Sarafianos’ lab figured out how to get the full picture: a detailed image of the unmodified proteins that filled all the gaps between hexamers.
The team used a technique called X-ray crystallography to unravel the protein’s secrets. Basically, they took many copies of the protein and coaxed them into forming a patterned, crystalline lattice. Next they shot high-powered X-ray beams at the crystal. By interpreting how the X-rays scattered when they ricocheted off the proteins, the researchers made a 3-D map of the protein.
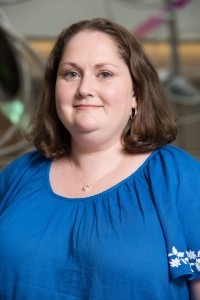
“But it doesn’t make sense until we make an atomic model of the protein to fit in that map,” said Karen Kirby, a research scientist at Bond LSC. “The map is just a grid that you can’t really interpret unless you put a model into it to see ‘Ok, it looks like this part is here, and that part is there, and this is how the protein is put together.’”
The researchers altered, tested and honed their 3-D model until it exactly matched the map produced by the X-ray diffraction pattern. This can be difficult and painstaking, but the researchers’ greatest challenge was creating the protein crystals in the first place: Scientists had been trying to crystallize the unmodified version of the HIV protein for decades without success.
To make a crystal, proteins are suspended in a liquid then slowly precipitated out, just like a “grow your own crystals” kit. But there are a lot of variables that control the process, from salts and additives in the liquid to the amount of protein in the mixture.
“It’s a very delicate balance to grow crystals,” Kirby said. “Many people call it more of an art than a science. It’s frustrating because you can never predict which solution will grow crystals. There are a large number of variables.”
Initially, most arrangements the researchers tried resulted in useless brown junk, Kirby said, caused by the proteins forming solids too quickly. Anna Gres, an MU chemistry grad student who led the project, used a crystallization robot to screen roughly 2,500 conditions.
That was the easy part, Gres said: “The real challenge begins afterwards, as one needs to manually optimize the initial crystallization conditions to find the one that will produce protein crystals of desired quality. This process can take years. In our case, I think we were lucky: It took approximately 500 manual screenings and about 6 month.” But the hard work paid off when she was finally able to produce lovely, hexagonal crystals. Surprisingly, the crystals formed in groups of six proteins, which matched their formation in the viral capsid.
The transition from tiny, useless particulate to invaluable crystals was tremendously exciting, Kirby said. But even to Kirby and Sarafianos, why their attempts succeeded when many others failed remains a little mysterious.
“I still don’t know what are the fine details that made the difference,” Sarafianos said.
“That’s the million dollar question,” said Kirby. “We really don’t have a good answer for that.”
Although solving the enigmatic crystal structure of the native full-length capsid protein was really rewarding, Gres said, she will continue to tinker with her technique: “I am still trying to optimize crystallization conditions, hoping to improve the quality of the crystals and diffraction.”
Water, water everywhere
Once the researchers got a good look at the interactions between hexamers, they were surprised by what they found.
Based on the genetic sequence of the protein, scientists speculated that they would be hydrophobic, or repel water. Instead, they found that “ordered” water molecules at specific sites played a crucial structural role by bridging interactions at the interface between hexamers.
“We thought, ‘How could these lowly waters really be of consequence?’” Sarafianos said. “But if you think about it, there’s 256 of these hexamers in the whole capsid and all kinds of interfaces among them: There’s thousands of water molecules that stabilize the whole structure. We hypothesize that this is an essential part of the stability of the whole capsid molecule.”
To test that hypothesis, they took the crystals, dehydrated them and checked to see if their shape changed. Although the protein lattices may look like sturdy crystals, they’re more like jello, Sarafianos said.
“The protein molecules are precariously touching each other and forming a lattice that is very, very sensitive. It’s held together in this case by water molecules in addition to other interactions.”
The change in shape suggested that water molecules are important in that they allow the capsid to assume different shapes. Moreover, Sarafianos said, the capsid’s malleability and plasticity could be critical to the life cycle of the virus and allow it to act as a multi-functional molecular Swiss army knife.
Onward with research
A clearer image of the capsid protein, could help Sarafianos’ lab gain a better understanding of how the body combats the virus and to discover new ways to disrupt the viral capsid.
“Now we have a system to study effects of capsid-targeting compounds with novel mechanism of action,” Gres said.
Working with a medicinal chemist, Sarafianos’ lab will undertake an iterative process of making compounds, solving their structures, testing them against HIV and then refining the molecules, with the ultimate aim of producing new and effective antiviral drugs.
Sarafianos is an associate professor of molecular microbiology and immunology and Chancellor’s Chair of Excellence in molecular virology with MU’s School of Medicine and an associate professor of biochemistry in the MU College of Agriculture, Food and Natural Resources.
The study, “X-Ray Crystal Structures of Native HIV-1 Capsid Protein Reveal Conformational Variability,” was recently published in the journal Science. Research funding has been provided by the National Institutes of Health (Grants AI112417, AI120860, GM103368, AI076119, AI099284, and AI100890 (SGS), and GM066087 (OP)). The content is solely the responsibility of the authors and does not necessarily represent the official views of the funding agencies.
Регистрация в binance
February 21, 2024 @ 2:25 pm
Thank you for your sharing. I am worried that I lack creative ideas. It is your article that makes me full of hope. Thank you. But, I have a question, can you help me? https://www.binance.com/ru-UA/join?ref=53551167
最佳Binance推荐代码
February 23, 2024 @ 8:56 pm
Your point of view caught my eye and was very interesting. Thanks. I have a question for you. https://www.binance.com/zh-CN/join?ref=P9L9FQKY
binance Inscreva-se
March 7, 2024 @ 3:12 am
Can you be more specific about the content of your article? After reading it, I still have some doubts. Hope you can help me.
бнанс акаунт
March 11, 2024 @ 3:28 am
Can you be more specific about the content of your article? After reading it, I still have some doubts. Hope you can help me.
Prihlásit se a získat 100 USDT
March 15, 2024 @ 5:31 am
Your article helped me a lot, is there any more related content? Thanks!
Kode Referal Binance
March 23, 2024 @ 12:29 pm
I don’t think the title of your article matches the content lol. Just kidding, mainly because I had some doubts after reading the article.
Registrasi Binance
March 24, 2024 @ 11:22 am
Thank you for your sharing. I am worried that I lack creative ideas. It is your article that makes me full of hope. Thank you. But, I have a question, can you help me?
registrace na binance
March 30, 2024 @ 4:09 am
I don’t think the title of your article matches the content lol. Just kidding, mainly because I had some doubts after reading the article.
https://mostbetcasino.blogspot.com/2021/10/explore-basics-of-gambling.html
April 3, 2024 @ 6:27 pm
When I originally commented I clicked the “Notify me when new comments are added” checkbox and
now each time a comment is added I get four e-mails witrh the same comment.
Is there any way you can remove me from that service?Bless you!
my page: https://mostbetcasino.blogspot.com/2021/10/explore-basics-of-gambling.html
Anonymous
April 14, 2024 @ 8:54 am
sex ロボット Oksexdollで同意できる合理的なセックスドールを購入するシリコンドールを請求する必要がある理由拡張されたセックスドールの信じられないほどの利点Irontechdollの新しい配置と新しい進歩
Anonymous
April 21, 2024 @ 9:36 pm
I don’t think the title of your article matches the content lol. Just kidding, mainly because I had some doubts after reading the article.